Журнал высшей нервной деятельности им. И.П. Павлова, 2022, T. 72, № 5, стр. 697-706
Meg study of somatosensory MMN evoked by electrical stimulation
O. E. Moiseenko a, *, D. O. Bredikhin a, M. Herrojo Ruiz b, V. V. Moiseeva a, A. N. Shestakova a
a Centre for Cognition & Decision Making, National Research University Higher School of Economics
Moscow, Russia
b Goldsmiths, University of London, Psychology Department
London, United Kingdom
* E-mail: moiseenko12olesya@gmail.com
Поступила в редакцию 8.03.2022
После доработки 12.04.2022
Принята к публикации 26.04.2022
- EDN: NKMNMI
- DOI: 10.31857/S0044467722050100
Аннотация
Abstract - Mismatch negativity (MMN) is a promising tool for studying the mechanisms of neuroplasticity, sensory memory, and other aspects of sensory function in health and disease. Despite numerous studies in the auditory modality, somatosensory MMN and its mechanisms remain under-examined. We employed “oddball” paradigm in which participants were presented with two blocks of standard (80%) and deviant (20%) stimuli to the second and fifth fingers of the right hand during video viewing. Permutation tests revealed significant differences between responses to standard and deviant stimuli in the condition associated with the perception of a standard stimulus by the second finger of the hand and a deviant stimulus by the fifth finger in the time window of 90–170 ms. We observed a fronto-central distribution of the MMN component, similar in spatiotemporal pattern to the somatosensory MMN known from the literature. Based on the results of our study, we consider it necessary to conduct additional studies to identify conditions in which the detection of MMN is reliable, as well as most comfortable for participants.
INTRODUCTION
The mismatch negativity (MMN) is a neurophysiological signal reflecting the brain’s automatic response to any discriminable change in the stimulation, traditionally auditory (for a review see Näätänen et al., 2007). It was first discovered in 1970-s and caught the attention of scientists as it could bridge basic and clinical research of early sensory perception (Näätänen et al., 2007). This signal can be evaluated with electroencephalography (EEG) or magnetoencephalography (MEG) by measuring the event-related responses or fields (ERP, ERF) locked to deviant stimuli embedded in a sequence of standard ones (Näätänen et al., 2007).
Initially, the auditory mismatch negativity received considerably more attention than its counterparts related to other sensory modalities. It has been extensively researched and appeared to be useful for assessing different clinical conditions, and it is the sole objective measure of the central auditory function (Kujala, Naatanen, 2010). Importantly, the MMN sheds light on the mechanisms by which the brain operates processing early sensory information and contributes to basic research. The MMN has also been studied in other sensory modalities such as visual (e.g., Wei et al., 2002; Astikainen et al., 2000; Näätänen et al., 2012; Näätänen et al., 2007; Kujala et al., 2017; Schwartz et al., 2018; Justo-Guillén et al., 2019), olfactory (Näätänen, 2007), and somatosensory (Akatsuka et al., 2005).
In the somatosensory modality, an important property of the MMN (termed somatosensory MMN or sMMN) is that it might be used to assess somatosensory discrimination in patients without their focused attention. This aspect is important given that certain populations might find it difficult to maintain attention on certain stimuli (e.g., pediatric population). Diminished somatosensory discrimination may be encountered in patients with developmental disorders, cerebellar lesions (Restuccia et al., 2006) or cervical dystonia (Chen et al., 2018). The developmental disorders with disturbed discrimination include but are not limited to dyslexia, autism, and developmental coordination disorder. Although the sMMN has been used far less than the auditory MMN to assess clinical conditions, investigating the sMMN has the potential to identify abnormal somatosensory plasticity in relevant patient groups, such as those listed above.
People with developmental coordination disorder might have motor difficulties and fail to perform motor skills which interfere with daily activities (Kirby, Sugden, 2007). A study by Job et al. (2019) addresses sensory integration in adults with probable developmental coordination disorder (pDCD). Participants were presented with sensory and tactile stimulation while executing delayed motor tasks. The results showed that participants with pDCD did not selectively process sensory inputs as healthy participants did. However, they were better and faster at selectively processing visual information. We suggest that this observation needs to be reliably confirmed and explored in other paradigms, e.g. somatosensory MMN. Cervical dystonia is another motor dysfunction that is also characterized by corrupted sensory discrimination. The mismatch responses elicited in this population appear to be abnormal. The interesting feature of the study done by Chen et al. (2018) is that they compared mismatch responses in two different modalities. In the auditory domain responses were similar to those obtained in the healthy population while in the tactile domain they differed. These results are consistent with the reports that there may be two different networks responsible for somatosensory and auditory integration (Chen et al., 2018). Patients with cerebellar lesions also show abnormal somatosensory mismatch responses (Restuccia et al., 2006). Moberget et al. (2008) investigated auditory MMN in patients with cerebellar degeneration and found that the results were inconsistent. Authors used four different types of deviants: for pitch and location there was no observed difference while for duration and intensity patients showed delayed MMN. Quintiliani et al. (2017) explored sMMN in patients with Dravet Syndrome that could be possibly linked to cerebellar abnormalities and also accompanied by deficits in visual and auditory sensory processing. Somatosensory mismatch responses in that group differed from the norm.
The novel use of sMMN was probed by the group of (Shen et al., 2017), who used it to investigate spatial factors in processing of sensory information and how the relative distance between body parts affects their representations in the cortex. The study employed the oddball paradigm and compared representations of the following body parts: a digit, the neck and a lip. Additionally, the authors aimed at comparing different digits of the hand. As a location for standard stimuli they used the lip in the first experiment and the second finger in the second. Other body locations were considered deviants. The results showed that responses elicited by deviant stimuli to different digits were significantly lower than those presented to the neck – for the amplitudes at different body locations the main effect was significant. As for the latency, there were no differences in it for the digits, but it was present in the case of other body locations. For example, latency was shorter for neck deviants in comparison with hand deviants. Based on their somatotopic findings, Shen et al., (2018a, 2018b) called sMMN a window to somatosensory representations in adults and infants. Indeed, it might turn out to be promising to employ sMMN as a tool for mapping of somatosensory cortical representations in healthy and clinical populations.
Due to the low number of studies employing sMMN, the choice of appropriate experimental paradigm seems to be highly important. Currently, there are a number of paradigms that are used to elicit sMMN: conventional “oddball” (Stefanics et al., 2014), “roving”, “standard-omitted” (Strömmer et al., 2014), and “optimal” (Näätänen et al., 2004). The most popular one is called an “oddball” paradigm. It accommodates two types of randomly interspersed stimuli: “deviant” or “rare” stimuli and “standard” frequently repeated stimuli. Although some studies employ a task-specific “active oddball” for eliciting P300, for eliciting MMN most studies employ a “passive oddball” that does not require voluntary attention from participants (Stefanics et al., 2014). A “standard-omitted” paradigm uses omissions instead of deviant stimuli (Strömmer et al., 2014). In a “roving” paradigm the “deviant” becomes “standard” over the course of time allowing for studying “repetition effects” (Stefanics et al., 2014; Shestakova et al., 2002). The “optimal” paradigm was invented by Näätänen et al. (2004) for eliciting mismatch negativity in the auditory modality. The authors tested if five different deviants comprising half of the stimuli could still elicit MMN. The obvious advantages of this paradigm are reduction of time and “profile for auditory discrimination abilities” obtained for different attributes (Näätänen et al., 2004).
Different types of stimulation can be used to elicit sMMN. Stimulation could be generated by an inflatable membrane (Andersen, Lundqvist, 2019; Shen et al., 2017), electromechanical vibrator (Kekoni et al., 1997), electromagnetic mechanical stimulator (Chen et al., 2018), constant current stimulator (Strömmer et al., 2014). Electrical stimulation could be delivered via electrode rings or solenoids (Restuccia et al., 2006, 2009; Job et al., 2019). Electrical stimulation seems to be the most conservative means to evoke sMMN. Moreover, electrical stimulation is routinely used in clinical practice of testing muscle excitability in amyoplasia patients (Agranovich et al., 2019) which as we can discuss later could be the recipients of the sMMN protocol we develop here using healthy adult controls.
In this study we aim at replicating previous sMMN results in the standard oddball paradigm using electrical stimulation and MEG, the neuroimaging technique that records magnetic fields produced in the brain. MEG is known for high temporal resolution, which renders it an optimal technique to assess MMN responses (for a review, see Naatanen et al., 2007). In addition, MEG is considered a safe and friendly technique for studying neuroplasticity in the clinical population.
Taking everything into account, we further aim to develop a specific protocol for probing sensitivity of peripheral muscles using electrical stimulation in order to later translate our protocol to clinical research using both EEG and MEG at the individual level.
METHODS
Participants. We recruited 20 healthy right-handed adults from 18 to 35 years old without self-reported neurological or psychiatric conditions of which 15 were women and 5 were men. The experiment was approved by the ethics committee of the National Research University Higher School of Economics. Informed consent was obtained from each volunteer.
MEG recording. The MEG data was collected using a 306-channel VectorView system (Elekta Neuromag, Finland) at the unique research facility ‘Center for Neurocognitive Research (MEG-Center)’ in MSUPE. The 306 sensors included 102 magnetometers and two sets of 102 planar gradiometers. We recorded MEG at a sampling rate of 1000 Hz. The hardware filters were set to AD-330 Hz. Four additional bipolar channels were used to record vertical electrooculogram (vEOG, left eye).
The 3-dimensional Fastrak digitizer (Polhemus, Inc, USA) was used to digitize the positions of the head position indicator coils, the landmarks of nasion, left and right preauricular points, and around 160 additional random points to obtain information about the head shape, which allows more accurate alignment with the anatomical MR images. The present study, however, did not use individual MRIs for further analyses. Two head position indicator coils were placed above the left and right eyes, as close as possible to the hairline, while another two were placed on the left and right mastoids respectively.
Stimulation. We used Digitimer High Voltage Stimulator model DS7A for tactile stimulation, following a previous study (Strömmer et al., 2014). The device was created for nerve and muscle tissue stimulation and provides brief pulses of high voltage. The width of the pulse was 200 μs. We used a staircase procedure to determine the individual participant’s sensory threshold. The sensory threshold was estimated separately for the second and fifth fingers. We did approximately 6 staircase rounds on each participant starting from the minimal intensity and increasing it in a stepwise manner (each time for 0.05 mA). The sensory threshold was the average threshold value from this procedure, which was then increased by 120% in intensity following Hautasaari et al. (2019). We then asked participants whether the obtained 120% threshold value would be comfortable for them during the experiment.
After the experiment, each participant was asked whether they felt the stimulation. They were also asked whether they concentrated on the stimulation, whether it changed throughout the experiment and how distracting the movie was.
Procedure. We employed the auditory “oddball” paradigm with approximately 500 stimuli (20% of deviants), interstimulus interval of 2000 ms and 200 μs as a width of the pulse. Stimuli were delivered to the second and fifth fingers.
In the first block (Block 1) 80% of stimuli (400) were delivered to the second finger and 20% (deviants, 100) – the fifth. After that, to avoid the “habituation effect” and to save time, participants were asked to sit still for 10 minutes. In the second block (Block 2), the pattern of stimulation was reversed: 80% of stimuli will be delivered to the fifth finger and the rest – to the second one.
Participants were instructed not to pay attention to stimulation, not to move excessively and watch a nature documentary with low sound level as electrical stimulation can be distracting. In a post-experimental briefing participants admitted that the chosen movie was distracting, and they sometimes had been forgetting about the applied stimulation. Participants were also instructed that they would be asked to report events of the movie after the recording.
Data analysis
The MEG analysis focused on magnetometer signals. Sensors and time segments contaminated with artifacts were first manually picked and removed. Next, we performed spatiotemporal signal space separation (tSSS) and movement compensation using the built-in in the Elekta software (MaxfilterTM; Elektra Neuroscience 2010). The subspace correlation threshold for tSSS was 0.900. Subsequent steps of signal preprocessing were performed in the Fieldtrip toolbox (Oostenveld et al., 2011) for MATLAB.
The data were downsampled to 250 Hz and power-line noise was removed applying a notch filter at 50 and 100 Hz. The artifacts such as heartbeats and eye movements were excluded using Independent Component Analysis (ICA, fastica). On average, we excluded 3 components across participants (ranging from 1 to 4).
We extracted epochs locked to the stimulus onset between – 100 and 500 ms. The pre-stimulus interval of each epoch was used for baseline correction. Epochs contaminated with excessive noise were rejected by visual inspection. The MMN waveform was defined as the difference of ERFs evoked by standard and deviant stimuli.
RESULTS
The results showed that magnetic responses to standard and deviant stimuli differed significantly in Block 1 (Fig. 1). A significant spatiotemporal cluster was found at the 90-170 msec time window in a left-lateralized fronto-central region of 22 magnetometers (P = 0.006, after FWER-control). Contrarily, no significant sMMN clusters were found in Block 2 (Fig. 2).
Fig. 1.
Results of Block 1 [Standard stimulus delivered to the second finger; Deviant stimulus delivered to the fifth finger] statistical analysis. Upper panel: mean ERF waveform across 22 magnetometers forming a significant spatio-temporal cluster (P = 0.006). Lower panel: t-values map; magnetometers forming a significant spatiotemporal cluster marked by yellow. Topographical maps are obtained using an 80 ms average window. Рис. 1. Результаты статистического анализа Блока 1 [Стандартный стимул подается на второй палец; Девиантный стимул подается на пятый палец]. Верхняя панель: усредненный МПСС по 22 магнитометрам, образующим значительный пространственно-временной кластер (P = 0.006). Нижняя панель: карта t-значений; магнитометры, образующие значительный пространственно-временной кластер, отмеченный желтым цветом. Топографические карты сделаны с усреднением активности в течение 80 мс.
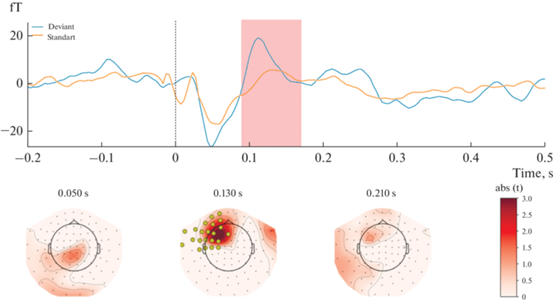
Fig. 2.
Results of Block 2 [Standard stimuli delivered to the fifth finger; Deviant ones delivered to the second finger] statistical analysis. Upper panel: mean ERF waveform across 22 magnetometers forming a spatio-temporal pattern of activation (P > 0.05). Lower panel: topographical t-values map. Topographical maps are obtained using an 80 ms average window. Рис. 2. Результаты статистического анализа Блока 2 [Стандартный стимул подается на второй палец; Девиантный стимул подается на пятый палец]. Верхняя панель: усредненный МПСС по 22 магнитометрам, образующим пространственно-временной паттерн активации (P > 0.05). Нижняя панель: карта t-значений. Топографические карты сделаны с усреднением активности в течение 80 мс.
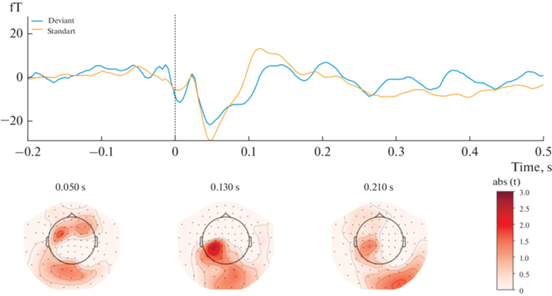
Sensory thresholds for each participant are presented in Table 1. The mean of the thresholds across participants in the second finger was 2.65 mA (SD = 0.4, SEM = 0.15) while in the fifth finger –1.96 mA (SD = 0.5, SEM = 0.16). Six participants asked to decrease the intensity of stimulation before the experiment (it was decreased by 0.03–0.1 mA). One participant asked to increase the stimulation (by 0.4 mA). At least three participants in the free discussion said that they felt stimulation less and less with the progression of the experiment.
Table 1.
Intensities of stimulation for each participant for each finger in mA Таблица 1. Интенсивность электрической стимуляции, подаваемой на второй и пятый палец, для каждого участника (мА)
Second finger | Fifth finger |
---|---|
2.6 | 2.75 |
2.5 | 1.9 |
3.9 | 3.3 |
3.0 | 1.65 |
2.5 | 1.25 |
1.3 | 1.6 |
3.5 | 2.4 |
2.35 | 0.9 |
3.0 | 1.15 |
2.5 | 2.25 |
1.85 | 1.85 |
2 | 1.2 |
2.4 | 1.9 |
3.3 | 3.2 |
3.5 | 3.0 |
1.9 | 1.1 |
2.2 | 1.45 |
3.75 | 2.55 |
2.5 | 2.35 |
2.35 | 1.45 |
DISCUSSION
We applied electrical stimulation in the oddball paradigm to evoke magnetic fields in response to a rare event interspersed between frequent ones. In particular, we recorded MMN responses in the condition when stimulation of the fifth finger was used as the deviant stimulus, and stimulation of the second finger was used as the standard stimulus (Block 1), and in the condition when stimuli were delivered in the opposite manner (Block 2). We observed a significant difference in responses to standard and deviant stimuli only in the condition of Block 1, but not in the condition of Block 2. We hypothesize that the absence of the sMMN in the latter case could happen due to the lesser intensity of stimulation of the fifth finger as compared to the second. It could be explained by inaccurate participants’ reports during the staircase procedure, or some other possible reasons: sponges might have dried out faster or/and were not securely attached to the fifth finger. Following this line of thinking, one can also explain sMMN results in the Block 1, where the electric stimulus to the second finger stimulation represents the standard stimulus while the omission of the stimulus to the same finger while stimulating the fifth finger could be considered as a deviant event.
Most studies reported sMMN in the time window from 100 ms to 200 ms after the stimulus onset as a negative or positive component. Other researchers reported MMN in the window of 150–250 ms when delivering stimulation in a variety of oddball paradigms. For example, Andersen and Lundqvist (2019) reported sMMN component at 125–145 ms in response to tactile stimulation paradigm delivered in “standard-omitted” fashion to the second finger. In a study using the same paradigm, Strömmer et al. (2014) reported sMMN at about 180–220 ms after the tactile stimulation onset in young adults. Importantly, the results revealed in our study indicate that the magnetic equivalent of the sMMN component could appear at 90–170 ms peaking at approximately 110 ms with.
The origin of this sMMN could stem from the activity over secondary somatosensory cortices as was previously suggested (Strömmer et al., 2014; Naeije et al., 2016, 2018). Importantly, sMMN is usually seen across the frontocentral regions of the brain and primary and secondary regions responding to sensory stimulation (Zhang et al., 2019). In the somatosensory modality, it can be recorded an area of central sulcus (Kekoni et al., 1997), and parieto-central and frontal regions contralateral to stimulated side (Restuccia et al., 2009). Notably, the results of our study showing contralateral fronto-central distribution of the evoked fields (see Fig. 1) are fully in accordance with the finding of Restuccia et al. (2009). Overall, both latency and topographies of sMMN recorded in our study corroborate previous sMMN findings in healthy adults obtained using tactile stimulation (Shen et al., 2018a).
We suggest that the differences in the spatio-temporal profiles of sMMN between the discussed studies might arise due to the variety of paradigms used to elicit the sMMN responses. For example, due to the fact that most sMMN studies do not report in detail what kind of techniques they used to determine an appropriate intensity of stimulation, the staircase procedure employed to define the stimulation intensity might turn out to be of a particular interest in terms of the effect consistency.
Another element of the paradigm, which we find important for the result interpretation, is the procedure used to eliminate non-MMN related activity. Taking into account the fact that MMN is an automatic and pre-attentive response, it is important to control that participants do not pay attention to stimulation, which allows for the elimination of responses related to active attention, such as the N2 and P3. In particular, participants might be asked to read a book or watch a movie (with no sound or low sound level). Interestingly, Strömmer et al. (2014) asked participants to listen to a radio play and answer questions about the contents afterwards to ensure their attention was focused on that task. At the same time, Mareau (2013) elicited aMMN during viewing the movie with sound, which suggests that such a procedure is possible. However, the MMN amplitudes recorded in the study by Mareau (2013) were reduced. Taking all the discussed approaches into account, we suggest that the use of a natural movie with low-level sound might be a fruitful compromise. Importantly, participants confirmed that the videos were sufficiently distracting to allow unattentive processing of somatosensory stimuli.
Moreover, we suggest that a consistent and user-friendly paradigm is especially important for the clinical population. Likewise, somatosensory processing could be impaired in a wide range of neurodevelopmental disorders (Cascio, 2010) and sMMN could be developed as an index of somatosensory processing integrity in clinical practice. In light of this, some researchers called sMMN a window to somatosensory representations in adults and infants (Shen et al., 2018a, 2018b) offering to use the sMMN as the means to study orderly somatotopic representations of body parts and fingers. Somatotopic patterns of MMN responses in infants as well as adults suggest that MMN could be sensitive to representations of body parts and the distance between these representations. Following the results of these studies, Shen et al. (2018a) proposed that MMN could be used to study typical and atypical development of somatosensory and motor systems, neural plasticity, representations of body parts and the boundaries between them. We further excel in sMMN investigations in the attempt to employ MEG approach to record evoked magnetic fields in the oddball paradigm without any contact with the surface of the skull. Potentially, MEG can provide exact locations of body-associated representations in healthy participants and patients.
Such an approach might turn out to be promising for a variety of musculoskeletal diseases such as arthrogryposis multiplex congenita (AMC). Despite the fact that arthrogryposis is considered to be a peripheral disorder, recent studies indicate that patients exhibit peculiarities of their brain signals at the resting level (Bhat et al., 2017; Blagoveschenskiy et al., 2018). Active elbow flexion in patients with AMC can be restored by the muscle autotransplantation of long-head triceps, latissimus dorsi transfer, pectoralis, and some other muscles to the position of biceps brachii (Oishi et al., 2017; Agranovich et al., 2019). However, patients might often experience difficulties with postoperative use of the muscle because the required neuroplastic reorganization might be complicated (Golosheykin et al., 2021). At the same time, the speed and quality of these processes are known to be associated with the choice of the donor muscle (Osichi et al., 2017). Therefore, the objective criteria for muscle choice are required in addition to donor muscle strength and its suitability for transfer. We suggest that the sMMN-based approach might become one of the objective neurophysiological markers for the assessment of the muscle’s suitability for autotransplantation. We plan to further use the current sMMN protocol to study sensorimotor plasticity in patients with arthrogryposis and use the obtained results or the in-between group comparison of pre- and postoperative results in sensory-motor function of this clinical group with the in the healthy population.
Overall, we suggest that sMMN pocesses certain advantages with regard to other methods for studying sensorymotor integration, such as two-point discrimination, detection threshold measurement, or behavioral measures. First, it does not rely on active attention which makes it very useful in populations for which it is hard to maintain attention. The technique also does not rely on subjective complaints and answers. Then, if methodology is strictly followed, might offer an objective assessment of sensorimotor integration processes. To control for this factor, we propose using staircase procedure to define a stimulation intensity and using nature videos with low sound effects to eliminate attentive processing of the somatosensory stimuli. In turn, we suggest that sMMN and its magnetic equivalent can be used to study the typical and atypical development of somatosensory and motor systems, neural plasticity, representation of body parts and boundaries.
Study limitations
One of the weaknesses of the research is that the majority of the participants reported feeling less stimulation on the second block of trials. That could possibly happen due to the technicalities such as the stimulator’s sponges drying, or the tape, which holds the stimulator in place, being too weak and getting loose by the end of the experiment, or both.
Another weakness would be electrical stimulation that is not wholly appropriate to our goals of working with children and clinical populations. Most studies on sMMN used tactile/mechanical/vibrotactile stimulators and electrical ones are not widely used.
Implications of using different types of stimulation were discussed in a study by Hautasaari et al. (2019). It has been already revealed that mismatch responses in the somatosensory domain could be elicited with different stimulation although most of the studies use electrical stimulation. The group decided to conduct two experiments using tactile and electrical stimulation, arguing that mechanical stimulation is more “natural” and less discomforting for a participant. As for the compatibility with neuroimaging methods, mechanical stimulator again is better suited because it produces less electromagnetic fields which could be disruptive for fMRI or MEG.
Список литературы
Благовещенский Е.Д., Агранович О.Е., Кононова Е.Л., Баиндурашвили А.Г., Назарова М.А., Шестакова А.Н., Габбасова Е.Л., Никулин В.В. Особенности электрофизиологической активности коры больших полушарий мозга у детей с артрогрипозом. Нервно-мышечные болезни. 2018. 8(2): 25–32. https://doi.org/10.17650/2222-8721-2018-8-2-25-32
Blagovechtchenski E., Agranovich O., Kononova Y., Nazarova M., Nikulin Vadim V. Perspectives for the Use of Neurotechnologies in Conjunction With Muscle Autotransplantation in Children Frontiers in Neuroscience. 2019. 13.https://doi.org/10.3389/fnins.2019.00099
Agranovich O.E., Kochenova E.A., Oreshkov A.B., Trofimova S.I., Petrova E.V., Gabbasova E.L., Blagovechtchenski E.D. Evaluation of unipolar transfer of the latissimus dorsi to flexor antebrachii in patients with arthrogryposis. Genij Ortop. 2019. 25: 42–48. https://doi.org/10.18019/1028-4427-2019-25-1-42-48
Akatsuka K., Wasaka T., Nakata H., Inui K., Hoshiyama M., Kakigi R. Mismatch responses related to temporal discrimination of somatosensory stimulation. Clinical Neurophysiology. 2005. 116(8): 1930–1937. https://doi.org/https://doi.org/10.1016/j.clinph.2005.04.021
Andersen L.M., Lundqvist D. Somatosensory responses to nothing: An MEG study of expectations during omission of tactile stimulations. NeuroImage. 2019. 184: 78–89. https://doi.org/https://doi.org/10.1016/j.neuroimage.2018.09.014
Cascio C.J. Somatosensory processing in neurodevelopmental disorders. Journal of neurodevelopmental disorders. 2010. 2(2): 62–69. https://doi.org/10.1007/s11689-010-9046-3
Chen J.-C., Macerollo A., Sadnicka A., Lu M.-K., Tsai C.-H., Korlipara P., Bhatia K., Rothwell J.C., Edwards M.J. Cervical dystonia: Normal auditory mismatch negativity and abnormal somatosensory mismatch negativity. Clinical Neurophysiology. 2018. 129(9): 1947–1954. https://doi.org/https://doi.org/10.1016/j.clinph.2018.05.028
Duncan C.C., Barry R.J., Connolly J.F., Fischer C., Michie P.T., Näätänen R., Polich J., Reinvang I., Van Petten C. Event-related potentials in clinical research: Guidelines for eliciting, recording, and quantifying mismatch negativity, P300, and N400. Clinical Neurophysiology. 2009. 120(11): 1883–1908. https://doi.org/https://doi.org/10.1016/j.clinph.2009.07.045
Golosheykin S.A., Blagoveschenskiy E.D., Agranovich O.E., Nazarova, M.A., Nikulin V.V, Moiseenko O.E., Chan R.W., Shestakova A.N. Feasibility and Challenges of Performing Magnetoencephalography Experiments in Children With Arthrogryposis Multiplex Congenita. In Frontiers in Pediatrics. 2021. 9. 1031. https://www.frontiersin.org/article/10.3389/fped.2021.626734
Hautasaari P., Kujala U.M., Tarkka I.M. Detecting differences with magnetoencephalography of somatosensory processing after tactile and electrical stimuli. Journal of Neuroscience Methods. 2019. 311: 331–337. https://doi.org/https://doi.org/10.1016/j.jneumeth.2018.09.014
Job X.E., Brady D., de Fockert J.W., Luft C., Hill E.L., van Velzen J. Adults with probable developmental coordination disorder selectively process early visual, but not tactile information during action preparation. An electrophysiological study. Human movement science. 2019. 66: 631–644. S0167-9457(18)30812-1. https://doi.org/10.1016/j.humov.2019.02.018
Justo-Guillén E., Ricardo-Garcell J., Rodríguez-Camacho M., Rodríguez-Agudelo Y., Lelo de Larrea-Mancera E.S., Solís-Vivanco R. Auditory mismatch detection, distraction, and attentional reorientation (MMN-P3a-RON) in neurological and psychiatric disorders: A review. International Journal of Psychophysiology. 2019. 146: 85–100. https://doi.org/https://doi.org/10.1016/j.ijpsycho.2019.09.010
Kekoni J., Hämäläinen H., Saarinen M., Gröhn J., Reinikainen, K., Lehtokoski A., Näätänen R. Rate effect and mismatch responses in the somatosensory system: ERP-recordings in humans. Biological Psychology. 1997. 46(2): 125–142. https://doi.org/https://doi.org/10.1016/S0301-0511(97)05249-6
Kirby A., Sugden D.A. Children with developmental coordination disorders. Journal of the Royal Society of Medicine. 2007. 100(4): 182–186. https://doi.org/10.1177/014107680710011414
Kujala T., Leminen M. Low-level neural auditory discrimination dysfunctions in specific language impairment—A review on mismatch negativity findings. Developmental Cognitive Neuroscience. 2017. 28: 65–75. https://doi.org/https://doi.org/10.1016/j.dcn.2017.10.005
Moberget T., Karns C.M., Deouell L.Y., Lindgren M., Knight R.T., Ivry R.B. Detecting violations of sensory expectancies following cerebellar degeneration: A mismatch negativity study. Neuropsychologia. 2008. 46(10): 2569–2579. https://doi.org/https://doi.org/10.1016/j.neuropsychologia.2008.03.016
Näätänen R., Kujala T., Escera, C., Baldeweg T., Kreegipuu K., Carlson S., Ponton C. The mismatch negativity (MMN) – A unique window to disturbed central auditory processing in ageing and different clinical conditions. Clinical Neurophysiology. 2012. 123(3): 424–458. https://doi.org/https://doi.org/10.1016/j.clinph.2011.09.020
Näätänen R., Paavilainen P., Rinne T., Alho K. The mismatch negativity (MMN) in basic research of central auditory processing: A review. Clinical Neurophysiology. 2007. 118(12): 2544–2590. https://doi.org/https://doi.org/10.1016/j.clinph.2007.04.026
Näätänen R., Pakarinen S., Rinne T., Takegata R. The mismatch negativity (MMN): towards the optimal paradigm. Clinical Neurophysiology. 2004. 115(1): 140–144. https://doi.org/https://doi.org/10.1016/j.clinph.2003.04.001
Naeije G., Vaulet T., Wens V., Marty B., Goldman S., De Tiège X. Multilevel Cortical Processing of Somatosensory Novelty: A Magnetoencephalography Study. Frontiers in human neuroscience. 2016. 10. 259. https://doi.org/10.3389/fnhum.2016.00259
Naeije G., Vaulet T., Wens V., Marty B., Goldman S., De Tiège X. (2018). Neural Basis of Early Somatosensory Change Detection: A Magnetoencephalography Study. Brain Topography. 2018. 31: 242–256. https://doi.org/10.1007/s10548-017-0591-x
Oishi S.N., Agranovich O., Pajardi G.E., Novelli C., Baindurashvili A.G., Trofimova S.I., Abdel-Ghani H., Kochenova E., Prosperpio G., Jester A., Yilmaz G., Şenaran H., Kose O., Butler L. Treatment of the upper extremity contracture/deformities. J Pediatr Orthop. 2017. 37: 9–15. https://doi.org/10.1097/BPO.0000000000001002
Oostenveld R., Fries P., Maris E., Schoffelen J.M. FieldTrip: Open source software for advanced analysis of MEG, EEG, and invasive electrophysiological data. Computational intelligence and neuroscience. 2011. 156869. https://doi.org/10.1155/2011/156869
Quintiliani M., Battaglia D.I., Restuccia D., Musto E., Perulli M., Contaldo I., Gambardella M.L., Palazzese G., Meloni A., Dravet C., Mercuri E., Guzzetta F. Somatosensory mismatch negativity in Dravet Syndrome. European Journal of Paediatric Neurology. 2017. 21(1): e143–e144. https://doi.org/https://doi.org/10.1016/j.ejpn.2017.04.1292
Restuccia D., Marca G. Della, Valeriani M., Leggio M.G., Molinari M. Cerebellar damage impairs detection of somatosensory input changes. A somatosensory mismatch-negativity study. Brain. 2006. 130(1): 276–287. https://doi.org/10.1093/brain/awl236
Restuccia D., Zanini S., Cazzagon M., Del Piero I., Martucci L., Della Marca G. Somatosensory mismatch negativity in healthy children. Developmental Medicine Child Neurology. 2009. 51(12): 991–998. https://doi.org/10.1111/j.1469-8749.2009.03367.x
Schwartz S., Shinn-Cunningham B., Tager-Flusberg H. Meta-analysis and systematic review of the literature characterizing auditory mismatch negativity in individuals with autism. Neuroscience & Biobehavioral Reviews. 2018. 87: 106–117. https://doi.org/https://doi.org/10.1016/j.neubiorev.2018.01.008
Shen G., Smyk N., Meltzoff A., Marshall P. Using somatosensory mismatch responses as a window into somatotopic processing of tactile stimulation. Psychophysiology. 2018a. 55: e13030. https://doi.org/10.1111/psyp.13030
Shen G., Weiss S.M., Meltzoff A.N., Marshall P.J. The somatosensory mismatch negativity as a window into body representations in infancy. International Journal of Psychophysiology. 2018b. 134: 144–150.
Shestakova A., Brattico E., Huotilainen M., Galunov V., Soloviev A., Sams M., Ilmoniemi R., Näätänen R. Abstract phoneme representations in the left temporal cortex: magnetic mismatch negativity study, NeuroReport. 2002. 13(14): 1813–1816.
Stefanics G., Kremláček J., Czigler I. Visual mismatch negativity: A predictive coding view. Frontiers in Human Neuroscience. 2014. 8: 666. https://doi.org/10.3389/fnhum.2014.00666
Strömmer J.M., Tarkka I.M., Astikainen P. Somatosensory mismatch response in young and elderly adults. In Frontiers in Aging Neuroscience. 2014. 6: 293. https://www.frontiersin.org/article/10.3389/fnagi.-2014.00293
Zhang Z., Guo G., Zhang J., Li C., Huang Q., Go R., Fukuyama H., Funahashi S., Yan T., Wu J. Do theta oscillations explain the somatosensory change detection mechanism? Biological psychology. 2019. 143: 103–112. https://doi.org/10.1016/j.biopsycho.2019.02.001
Дополнительные материалы отсутствуют.
Инструменты
Журнал высшей нервной деятельности им. И.П. Павлова