Журнал высшей нервной деятельности им. И.П. Павлова, 2021, T. 71, № 4, стр. 485-499
Functional Near-Infrared Spectroscopy Applications in Developmental Cognitive Neuroscience
M. A. Sitnikova a, b, *, S. B. Malykh c, **
a Research and Project Centre for Cognitive Neuroscience and Neurotechnologies, Belgorod National Research University
Belgorod, Russia
b Russian Academy of Education
Moscow, Russia
c Psychological Institute of Russian Academy of Education, Russian Academy of Education
Moscow, Russia
* E-mail: furmanchuk@bsu.edu.ru
** E-mail: malykhsb@mail.ru
Поступила в редакцию 22.11.2020
После доработки 22.12.2020
Принята к публикации 22.12.2020
Аннотация
Functional near-infrared spectroscopy (fNIRS) is known as a versatile and much promising non-invasive neuroimaging method extensively used nowadays in developmental cognitive neuroscience for measuring neural substrates underlying mental activity and processes involved in cognition, social interaction, and learning over the life span. This paper focuses on a summary of the basic principles of fNIRS technique concerning its design, implementation, and measurements. A detailed review of fNIRS strengths and benefits over other neuroimaging modalities is provided. One of the aims is to illustrate the fNIRS advantages by findings of recent studies from the wide variety of fNIRS applications: language, numerical cognition, executive functions, emotions, memory, motor functions, with a particular focus on neuroimaging in naturalistic environments. Moreover, the overview of recent implementations as well as future perspectives of fNIRS application in accordance with current trends in developmental sciences domain is considered: hyperscanning (simultaneous multi-subject measurements); multimodal measurements (combining several neuroimaging modalities in one research); neurofeedback trainings for enhancing cognitive functions in children and adults; use of interactive immersive stimuli and virtual reality (VR) in developmental cognitive neuroscience. Important limitations and challenges of fNIRS within healthy individuals in daily life settings are highlighted, as well as possible technical solutions and methodological procedures of their overcoming are discussed.
INTRODUCTION
The insight in many developmental questions covering maturation of perceptual, cognitive and motor functions from birth to adulthood, age-related differences in behavior and social interaction, trajectories of typical and atypical development, and mechanisms that facilitate and enhance learning during certain periods in development can be expanded significantly by up-to-date neuroimaging findings available in neuroscience. Developmental cognitive neuroscience (Munakata et al., 2004) can be considered a prominent multidisciplinary research field involving neuroscience, developmental and cognitive psychology, social and educational science. The main research questions of developmental cognitive neuroscience are to understand the underlying mechanisms of cognition in the brain over the life span or age-related changes in behavior; to trace how these cognitive functions change throughout development and learning, on the one hand; what are new beneficial perspectives for education and how to maximize human potential in learning and knowledge acquisition applying neuroscience evidence, on the other hand.
There can be great developmental changes on the neural level in comparison to changes at the behavioral level (Morita et al., 2016) proving that similarity in behavior can be the result of activation of different neural networks in processing the cognitive or social information. With the introduction of neuroimaging methods into cognitive neuroscience practice and the rapid advancement of functional brain imaging techniques, scientists were given an opportunity to identify the neural correlates of a wide variety of cognitive functions, from perception to higher-order mental activities (Cutini et al., 2012). Recently appeared social neuroscience as well as “real-world neuroscience” (Matusz et al., 2019) or “interactive social neuroscience” (Minagawa et al., 2018) have enabled developmental cognitive neuroscience move further towards the experiments in naturalistic environment to focus on how brain reflects and mediates interactions in real-life situations with two or more participants, their social relations, social cognition. Therefore, nowadays brain regions activated while people at different age stages acquire competencies and perform simple or complex mental tasks can be investigated within classic laboratory research, “naturalistic” laboratory research and emerging recently a “fully naturalistic” real-world research (Matusz et al., 2019).
Over the last 30 years, the emerging of new and promising neuroimaging techniques has been used to unveil the structure and functions in the human brain and to shed light on the relationship between activity in certain areas of working brain and mental activity in humans (Lauritzen, Gold, 2003). The most prominent and widely used functional neuroimaging modalities are functional magnetic resonance imaging (fMRI), electroencephalography (EEG), magnetoencephalography (MEG), positron emission tomography (PET), and functional near-infrared spectroscopy (fNIRS). Each of them has its advantages and limitations in various domains of developmental cognitive neuroscience. In this paper, we’ll discuss in detail technical and methodological aspects, as well as current perspectives of applications of functional near-infrared spectroscopy (fNIRS) in cognitive science, developmental psychology, education, social relations, and interaction.
FNIRS as a valuable, versatile and non-invasive vascular-based neuroimaging technique for measuring brain hemodynamic was introduced around 40 years ago. For functional brain mapping it’s been applied since the early 1990s (Cutini et al., 2012; for pioneering works see Villringer et al., 1993; Chance et al., 1993; Hoshi, Tamura, 1993). For more than 25 years the number of fNIRS publications has increased tremendously and nowadays continues to grow rapidly due to technological developments, data analysis advances, and novel areas of application covering a wide range of topics in developmental, educational and social neuroscience domains (Fantini et al., 2018; Blasi et al., 2019). fNIRS has been stated as a feasible and much promising technique for the exploration of the neural substrates underlying cognitive functions, mental activity and processes involved in educational interaction and learning: language acquisition, reading, numerical cognition, calculation, math development, sensory and motor functions, emotions, memory, executive functions, attention.
As powerful tools, neuroimaging techniques, in particular functional near-infrared spectroscopy (fNIRS), has complemented traditional behavioral paradigms and assessment methods available in psychological and pedagogical approaches (Howard Jones et al., 2016) and provided reliable measures to understand on the neural level the typical and atypical trajectories of development, and the effects of educational interventions on them (Ansari et al., 2012; Vanderwert, Nelson, 2014) with consideration of both person-dependent and situation-dependent social factors (Shamay-Tsoory, Mendelsohn, 2019).
BASIC PRINCIPLES OF FUNCTIONAL NEAR-INFRARED SPECTROSCOPY
Functional near-infrared spectroscopy (fNIRS), diffuse optical imaging (DOI), diffuse optical tomography (DOT), optical topography, NIR imaging are the synonymous names for the neuroimaging technique where changes in relative concentrations of oxygenated and deoxygenated hemoglobin are measured in brain cortex by using near-infrared light. The main principles of this technique are based on (1) neurovascular coupling, (2) near-infrared light propagation in the brain, and (3) absorption of the light by the main chromophores (HbO and HbR) (for more in-depth reviews see (Cutini et al., 2012; Scholkmann et al., 2014; Pinti et al., 2020; Curtin, Ayaz, 2018; Quaresima, Ferrari, 2019a, 2019b)).
fNIRS doesn’t reflect neuronal activity directly, it’s an indirect method that monitors changes in tissue hemodynamics (blood perfusion) in response to brain activation, on the basis that neural activation in a distinct brain area and vascular response are tightly coupled (León-Carrión, León-Domínguez, 2012; Scholkmann et al., 2014). A typical activation revealed by fNIRS in a cerebral cortex area is sketched in Fig. 1. An increase in neural activity is accompanied by an increase in regional cerebral blood flow (CBF), providing glucose and oxygen that are not present in neurons, but important for metabolism, to the area of active neurons. Thus, neurovascular coupling assures an increase in CBF in a temporally and spatially coordinated manner in response to changes in neural activity through a complex sequence of coordinated events involving neurons, glia, and vascular cells, and signaling molecules (Quaresima, Ferrari, 2019a).
Fig. 1.
The increase in HbO and the concomitant relatively smaller decrease in HbR reflect changes in brain hemodynamics in response to neural activity.
Рис. 1. Увеличение насыщенного кислородом гемоглобина (HbO) и одновременное относительно небольшое снижение обедненного кислородом гемоглобина (HbR) отражают изменения гемодинамики мозга в ответ на нейронную активность.
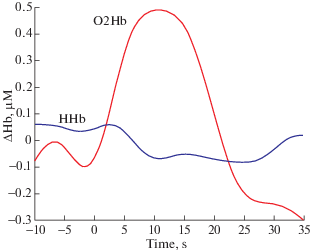
Optical imaging has been successfully used to create human brain maps to visualize the stimulated areas in task-related brain activation studies within cognitive, social and educational neuroscience. A brain function can be determined by measuring the difference in oxygenated (HbO) and deoxygenated (HbR) hemoglobin concentrations at a baseline and at task performance that displays an increase or decrease in CBF in response to brain activity in the corresponding cortical region. This states for the specific spatial correlation between CBF and any cognitive or motor task performed (León-Carrión, León-Domínguez, 2012). As for the temporal correlation, there is a temporal delay of 1–2 seconds in hemodynamic response to neural activation, with peaks at around 4–6 seconds after the neural response. It can be considered one of the most severe scientific limitations of this technique imposed by neurovascular coupling.
Light from the near-infrared range has the ability to penetrate biological tissue (e.g., skin, skull, brain) and is mainly absorbed by oxygenated and deoxygenated hemoglobin. Typically, for studying cognitive functions two specific wavelengths are chosen within the light spectrum range of 650–900 nm – the so-called biological “optical window” (Fig. 2), when light-absorbing molecules (chromophores) HbR and HbO are mobilized and their concentration changes can be easily detected (Jöbsis, 1977, Delpy et al., 1988).
Fig. 2.
Absorption spectra for HbO and HbR for near-infrared wavelengths of 650–900 nm. Reproduced from nirx.net.
Рис. 2. Спектры поглощения HbO и HbR для волн длиной 650–900 нм в ближнем инфракрасном диапазоне (БИК-диапазоне). Воспроизведено с сайта nirx.net.
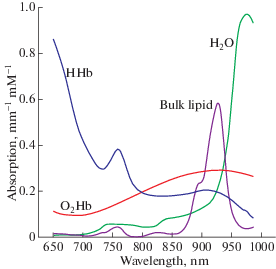
The current standard technology is represented by multichannel fNIRS devices composed of multiple optodes placed on the scalp: light-emitting diodes (LEDs) or laser sensors, that send NIR light, and photodetectors, that capture light waves after they have traveled through the brain tissue. Locations of emitters/detectors can be chosen using neuronavigation systems. A pair of emitter-detector optodes forms a measuring channel. The trajectory of photons traveling from the emitter to the detector is represented by a “banana-shaped” form so that the emerging light in part pervades cortical tissue from 1.5 to 2.5 cm. In general, NIR light penetration depth depends on (1) the light scattering tissues, and (2) absorption qualities of chromophores (HbO and HbR) at different wavelengths, and on (3) the separation distance between the light emitter and detector (the depth is around half of the emitter-detector physical distance) (Quaresima, Ferrari, 2019a). Most commonly used in cognitive neuroscience a source-detector separation distance is 25–30 mm (in adults) and 40–50 mm (in infants) to achieve a balance between signal-to-noise ratio and to provide enough depth to reach the most superficial layers of the cerebral cortex (Strangman et al., 2013; Brockington et al., 2018). fNIRS allows simultaneously measure oxygenated, deoxygenated and total hemoglobin (the sum of HbO and HbR) concentration changes with high temporal resolution from 1 to 100 Hz (typically > 10 Hz), calculated by using the modified Lambert-Beer law.
Mostly used nowadays fNIRS systems are continuous-wave (CW), where near-infrared light is continuously sent from emitting optodes through brain tissue to detecting optodes. fNIRS devices are available in different modifications and specifications: from high-density multi-channel systems to portable, ultra-light, freely configurable and battery-operated fNIRS instruments comprising of a small number of illumination sources and detection sensors. The choice of the configuration of the instrumentations depends on the aims of the research. To get a clear understanding of perspectives and potential pitfalls of fNIRS applications in cognitive and social neuroscience, and neuroeducation, fNIRS advantages over other methods as well as limitations will be highlighted in the next section.
ADVANTAGES AND LIMITATIONS OF FNIRS TECHNIQUE
fNIRS has been often considered as a complementary technique that can offer some important, but additional knowledge to the one received by well-known and often used methods such as EEG and fMRI.When comparing and contrasting fNIRS with other neuroimaging modalities, one should pay attention to such criteria as temporal resolution, spatial resolution, and the degree of immobility.
First, we would like to point out at important advantages of fNIRS:
– relatively high temporal resolution (Pinti et al., 2020; Quaresima, Ferrari, 2019b). FNIRS is a little slower than EEG, but much faster than fMRI and PET;
– acquiring data about relative concentration changes in HbO and HbR. Both fNIRS and fMRI are functional neuroimaging techniques based on the neurovascular coupling. However, fMRI captures only based on the blood-oxygen-level-dependent (BOLD) signal that relates to HbR changes. In fNIRS HbO can be additionally measured “providing a more complete evaluation of the cortical hemodynamic response” (Quaresima, Ferrari, 2019b);
– a relatively low-cost technique;
– whole brain coverage with high-density devices. Nowadays high-density diffuse optical tomography (HD-DOT) technological solutions enables to get up to 128 measurement channels and tremendously upgrade spatial resolution and diminish the influence of contaminations (Eggebrecht et al., 2014);
– the user-friendly technique, safe (no harmful radiation because of NIR light) and easily applicable in studies with newborns (Liao, Culver, 2014), infants (Urakawa et al., 2015; Aslin et al., 2015; Wilcox, Biondi, 2015), children (Nagamitsu et al., 2012). Participants can move comfortably during an experiment, and no gel or other liquid needs to be added to their head to improve optode-scalp contact, as in EEG. No substance is necessary to inject, and no safety concerns such as gamma radiation in PET;
– no severe motion restrictions and body movements – subjects can be measured in natural upright and sitting position (Balardin et al., 2017). In developmental cognitive neuroscience, the natural sitting position is preferable to concentrate on solving mental tasks, especially if measuring brain activation in school children of different age ranges. Moreover, low sensitivity to motion artifacts allows investigating embodied cognitions (Bahnmueller et al., 2014). In fMRI and PET, on the contrary, a subject is in a supine position and therefore hemodynamic changes differ from those in sitting or standing positions (Quaresima, Ferrari, 2019b);
– freely moving during the fNIRS measurements with wireless and wearable devices. It enables application during an outdoor activity in real-life situations like walking paradigm, for example (Pinti et al., 2015; Schneider et al., 2014; Herold et al., 2017). Mobile fNIRS devices, as well as mobile EEG systems, both used for real-time monitoring of brain activity, have become popular and well-established in applied neuroscience nowadays (Pinti et al., 2015; Bleichner et al., 2015). “Their miniaturization does not interfere with capturing active behavior in social environments” (Quaresima, Ferrari, 2019b);
– transportability/portability that provides applicability of fNIRS in natural settings. Scientists can bring “the lab” and neuroscientific research to schools, kindergarten, offices, hospitals, shopping malls and so on. Emerging of wearable fNIRS devices, on the one hand, and fNIRS paradigms derived from real-world educational settings or social interactive environment, on the other hand, have opened a new trend in developmental cognitive neuroscience research of assessing neural correlates of cognitive function and dysfunction in realistic scenarios, that are objectively more accurate in than lab-based research (Pinti et al., 2015; Brockington et al., 2018). Moreover, skepticism amongst researchers about the feasibility of using laboratory results obtained from the use of neuroimaging techniques, such as fMRI, PET, MEG or non-portable EEG to pedagogy and social science is preserved;
– high experimental flexibility if compare to the other neuroimaging methods combined with a silent measurement procedure. FNIRS ensures comfortable environment with no noise interference and much variety of possible responses (for example, by pressing a button, by writing an answer, by using an overt speech) in solving educational and social tasks to effectively study emotions and different cognitive functions: memory attention, language, and arithmetic (Suda et al., 2010; Soltanlou et al., 2018b). Subjects may play games or be occupied in social activities on the computer or with an experimenter (Brockington et al., 2018; Quaresima, Ferrari, 2019b). Other neuroimaging modalities can’t offer such a great variety of research paradigms because of some important restrictions: for example, overt speech production and its neural correlates cannot be investigated by EEG, PET or fMRI due to their high sensitivity to movement artifacts, or a loud noise inside the MRI scanner may lead to such problems as math anxiety, cognitive load, and sensory input interference, especially with auditory stimuli;
– measurements of large samples of participants, using repeatedly or in a continuous manner for long-term monitoring purposes, for example, a long-term 7-day continuous monitoring of the neonatal brain (Galderisi et al., 2016), or 4-hour monitoring of an adult while carrying out his daily activities (Balardin et al., 2017). In general, an fNIRS measurement lasts from 30 to 60 minutes, with a possibility of a short rest between tasks;
– feasibility of hyperscanning (multi-subject measurements) to provide neural underpinning of cognitive and emotional processes in realistic social interactions (Cui 2012; Babiloni, Astolfi, 2014; Cheng et al., 2015; for reviews, see Koike et al., 2015; Scholkmann et al., 2013). Such neuroimaging methods, like fMRI, MEG, PET can be potentially used in hyperscanning research in lab-based experiments only, no real social interaction experiments with two or more simultaneously applied brain imaging devices are possible. By contrast, the hyperscanning approach in fNIRS studies seizes moment-to-moment interactions in a natural context, for example during natural verbal communication between storytellers and listeners (Liu et al., 2017), face to face perception (Suda et al., 2010);
– multimodal measurements combining fNIRS with such neuroimaging modalities as fMRI (Funane et al., 2015; Scarapicchia et al., 2017), MEG, EEG (Chen et al., 2016), eye-tracking (Urakawa et al., 2015), tDCS, TMS (Curtin et al., 2019) for investigating the brain at multiple spatial and temporal scales simultaneously;
– neurofeedback (Kohl et al., 2019) applications to facilitate human communication and interaction with the environment by directly measuring and self-regulating the hemodynamic activity in the brain.
Among the most important and pronounced limitations of fNIRS for human brain studies are:
– a limited penetration depth (only upper cortical regions within 1–1.5 cm). Mostly only cortical regions beneath the scalp can be reliably measured by fNIRS (Patil et al., 2011). Subcortical and deeper cortical regions, such as basal ganglia and amygdala cannot be investigated by fNIRS;
– a relatively low spatial resolution (around 1 cm). In fNIRS technique, there is no apparent single “spatial resolution”, as light absorption depends on the geometry of the sources-detectors array and the separation distance between them, where individual photon’s paths are integrated according to “banana shape” (Almajidy et al., 2020). One of the possible solutions to enhance spatial resolution is to enlarge the number of optodes on the scalp with various source-detector separation distances “providing overlapping sensitivity volumes” (Yücel et al., 2017). This approach is generally referred to as Diffuse Optical Tomography (DOT).Therefore, high density (HD) DOT has demonstrated accurate mapping of brain function with fNIRS, making its spatial resolution close to that of fMRI (Chitnis et al., 2016) for cortical regions close to the skull;
– fNIRS doesn’t provide anatomical information and structural images that can be used to interpret the activation patterns. To settle the spatial localization of the cortical hemodynamic response and brain areas beneath the fNIRS probes it’s necessary to use either three-dimensional computerized MRI atlases or Montreal Neurological Institute (MNI) stereotactic coordinates of fNIRS measurements (Pinti et al., 2018);
– a strong sensitivity to extracerebral contaminations (for more in-depth information see Leff et al., 2011; Tachtsidi, Scholkmann, 2016; Scholkmann et al., 2014). The task-evoked hemodynamic response includes not only systemic blood flow changes due to neurovascular coupling, but also perfusion due to extracerebral (scalp) blood flow, blood pressure, sympathetic activation or psychophysiological influences (Pfeifer et al., 2018) that interferes with an accurate estimation of the stimulus-evoked responses in the brain (Yücel et al., 2017). One of the best possible solutions to eliminate the signal contamination by task-evoked hemodynamics, not due to neurovascular coupling is short-distance/multi-distance measurements (Brigadoi, Cooper, 2015). Such measurements allow separating signals coming from extracerebral layers of the head (via applying short distance detectors, with 5–10 mm source-detector separation) from the desired neurovascular coupling-related signals coming from the brain (via long source-detector separation channels, with 30–40 mm separation) (Fig. 3);
Fig. 3.
Multiple source-detector distances within the “banana-shaped” form of fNIRS signal. Reproduced from (Rupawala et al., 2018).
Рис. 3. Несколько вариантов расположения источников и детекторов на разном расстоянии друг от друга в соответствии со схемой сигнала fNIRS “банановидной” формы. Воспроизведено из (Rupawala et al., 2018).
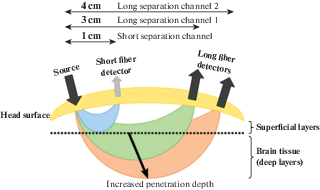
– response delay to the stimuli. The typical hemodynamic response function is characterized by a slower profile in comparison to that of neural activity: usually, hemodynamic activity begins to increase after about 1 s following changes in neural activity, it reaches its peak in around 5–7 s after neural activity, and it slowly returns to baseline activity after 12–15 s;
– susceptibility to ambient light (Almajidy et al., 2020) in a real-life environment. Due to the characteristics of the optodes placed into a cap, there is a minimal gap between emitter and skin, therefore some precautions are necessary to avoid ambient light influencing measurements (Orihuela-Espina et al., 2010), such as the proper emitter placement, spring holders for optodes to fix them tightly to the skin or additional external cap for outdoor studies.
fNIRS has firmly established its role as a neuroimaging tool especially under circumstances in which other methods fail. Specifically, fNIRS enables fully naturalistic experiments.
FNIRS APPLICATIONS IN DEVELOPMENTAL SCIENCES DOMAIN
FNIRS is nowadays a popular technique in the brain development domain by virtue of its easy, safe and user-friendly applicability for neuroimaging in newborns, infants, toddlers, children, and adolescents. The main characteristics that made fNIRS a key tool in infant and children neuroimaging research are no severe motion restrictions, no noise interference in combination with thinner scalp and skull in young children compared to adults (Boas et al., 2014; Vanderwert, Nelson, 2014; Wilcox, Biondi, 2015; Azhari et al., 2020). The most studied cognitive functions assessed by fNIRS are language acquisition, numerical cognition, spatial associations, executive functions, facial processing. In some studies, a pre-recorded social stimuli (stimuli that are relevant to real-life social interaction) are used, whereas some studies investigate infant brain responses to live social stimuli (a real actor talking to or playing with infants and young children) (Mcdonald, Perdue, 2018). The comprehensive reviews of various aspects of cognitive development in infants, preschoolers and children one can get in (Azhari et al., 2020; Fiske, Holmboe, 2019; Aslin et al., 2015; Nagamitsu et al., 2012; Lloyd-Fox et al., 2010). We provide several examples to illustrate the fNIRS applications in developmental neuroscience.
FNIRS has been successfully applied to assess brain neural networks for executive functions. Inhibitory control (IC) refers to the process of preventing an automatic or prepotent response to achieve a goal. Mehnert and colleagues (Mehnert et al., 2012) employed fNIRS to measure the neural substrates of IC in children aged 4–6 years compared to adult participants, using the Go/No-Go task. It was found that whilst the right frontal and parietal regions were activated in adults during No-Go (inhibition) trials, children maintained a high level of right frontal and parietal activation in both Go and No-Go trials, indicating the high inhibitory demand of the task. Another recent fNIRS study by Moriguchi and Shinohara (Moriguchi, Shinohara, 2019) used a more emotionally charged inhibitory control task, the “Less Is More” (LIM) task, with 34-year-olds. Stronger right inferior frontal cortex (rIFC) activation was found when children were able to inhibit pointing to the larger reward.
Another complex cognitive ability to understand or to interpret other people' beliefs, intents, desires, emotions, knowledge and to predict their behavior, known as the theory of mind (ToM), has been recently examined with fNIRS in infants (Hyde et al., 2018). 7-months-old infants showed significant activation in temporal-parietal junction (TPJ), but not in other temporal and frontal regions, while they viewed video scenarios of a person searching for a hidden object when this person’s belief about the location of the object was false. The results illustrated the involvement of TPJ in high-level social cognition already by around 7 months of age.
The robustness of fNIRS to muscle and head movements in case of reading and speaking is considered a significant advantage in neuroimaging studies of language development of children (Soltanlou et al., 2018b). Thus, the results of brain activation during three different reading tasks (silent reading, reading out loud, and free speech) in children and adults showed that the highest activation could be seen during a free speech in bilateral frontal regions (Tellis, Tellis, 2016). The sensitivity of newborns and infants to native and non-native languages was investigated by (Pena at al., 2003; Arimitsu et al., 2011; Vannasing et al., 2016). Full-term newborns showed larger neural activation in the left temporal area in response to native language compared to non-native language and non-linguistic stimuli (backward speech) or silence (Pena at al., 2003; Vannasing et al., 2016).
A series of studies was conducted by the Lloyd-Fox and her colleagues (2013, 2014, 2016). They found localized brain activation patterns in regions of the posterior superior temporal, anterior temporal and inferior frontal cortex in response to dynamic social cues (human-generated visual or auditory stimuli) in the UK and then in a Gambian cohort of infants in more naturalistic settings (not in a neurocognitive laboratory specially equipped for neuroimaging). These studies proved fNIRS to be a portable, suitable in the field technology within the developmental cognitive neuroscience domain.
FNIRS APPLICATIONS IN INTERACTIVE SETTINGS AND REAL-WORLD SOCIAL NEUROSCIENCE
fNIRS has been extensively and successfully used in laboratory settings within educational, developmental (Lloyd-Fox et al., 2010), and cognitive neuroscience (Cutini et al., 2012; Pinti et al., 2020) studies. Advantages of this technique enable to trace changes in brain morphology, long-range connectivity and activity as people learn and interact, to track safely and map in detail changes in cortical activation following extensive learning in the same individual, and to compare such changes with those observed in younger versus older children, or children versus adults. However, testing social perception and interaction in a realistic environment with fNIRS is only gaining popularity (Pinti et al., 2020; Brockington et al., 2018; Reindl et al., 2018; Liu et al., 2017). It can shed light on brain-to-brain coupling during interaction in a natural communicative context, on the one hand, or the process of acquiring knowledge, on the other hand, that are relevant in the real world.
In their research, Brockington and his colleagues (Brockington et al., 2018) presented some paradigms to explore real classroom activities combined with multi-subject measurements. In the teacher-student interaction experiment, both subjects underwent fNIRS hyper scanning whilst playing an educational board game in which the teacher aimed to explain the addition of two natural numbers to a child. Hemodynamics within the prefrontal cortex (PFC) in a child (involved in decision making and processes of high order cognition (calculating)), and temporo-parietal junction (TPJ) in a teacher (involved in social functions such as empathy and mentalizing) were studied during the experiment. The activation pattern in PFC of a child was positively correlated with the teacher’s anterior TPJ activation proving the alignment of neural activity between child and teacher during a naturalistic educational interaction. In another paradigm, a group activity of four students listening to a lecture was studied (Brockington et al., 2018). Sustained attention is known to be an important component of scholastic achievement (Steinmayr et al., 2010) and PFC plays a crucial role in mediating it on the neural level. Therefore, hemodynamic signals of four subjects were simultaneously monitoring during a lecture to measure the synchronicity of their brain activity. The findings showed an increase in activation in bilateral PFC in all subjects only during the first out of four lecture blocks indicating interbrain coupling related to students' broader attention span at the beginning of the lecture.These results can be helpful to improve teaching practices so that sustain attention is used for a more extended period during the classroom activities (Brockington et al., 2018).
The social interaction of two adults while playing a computer-based game was studied in (Cui et al., 2012). The coupling in brain activation measured in superior frontal cortices increased significantly during cooperation, but not during competition. Another example of investigating brain-to-brain coupling with fNIRS is the study of the social brain during verbal communication in natural settings between storytellers telling an unrehearsed real-life story and a group of listeners (Liu et al., 2017). This multi subjects’ study enabled two lines of analysis of neuroimaging data: listener-listener and speaker-listener neural coupling. A significant speaker-listener temporal coupling (with 5-s delay) was observed only during successful verbal communication (native language for listeners). During listening to a foreign language, communication was blocked, there was no neural synchronization detected. PFC activation in the speaker was significantly correlated with parietal areas in the listeners. The inter-subject activity evoked by the same story was reliable across the listeners (listener-to-listener coupling).
One of the recently available fNIRS applications is experiments with freely moving participants. Due to wearable and wireless configurations of fNIRS devices, the feasibility of measuring brain hemodynamics in response to cognitive tasks performed while subjects walking, doing sports, playing musical instruments outside the laboratory and in everyday life situations has been demonstrated (Balardin et al., 2017; Pinti et al., 2015). Here we provide several examples to illustrate this novel fNIRS advantage. Pinti and her colleagues (Pinti et al., 2015) explored the use of fNIRS to monitor brain activity during a memory task, counting objects in real-life situations, for example, doorbells, or perform a mental arithmetic task while walking around the city. Worth noting that the fNIRS system in this experiment proved to be robust against sunlight and motion artifacts, for example, extensive head or body movements.
In other experiments, cognitive processes together with a relatively moderate physical activity of one person (playing table tennis, or playing piano) (Balardin et al., 2017), or two people interacting (playing violin) (Vanzella et al., 2019) were studied. Playing music, or doing sports are highly sophisticated activity involving complex motor, cognitive, and social processes. The investigation of the brain underpinnings of joint musical actions revealed leader-follower relationships in musical ensemble performance in a naturalistic paradigm: greater activation in sensorimotor and temporo-parietal areas during the duo condition in comparison to solo in a musician with a follower role in duet (Vanzella et al., 2019).These studies illustrated a successful application of fNIRS in scenarios assessing synchronization during social interactions and daily activities where people were moving freely.
FNIRS APPLICATIONS IN MULTIMODAL MEASUREMENTS
Multimodal measurements have been gaining popularity nowadays for its potential to apply the advantages of two or more techniques to get evidence-based findings. Multi-modal integration is based on combining multiple neurophysiological signals: local hemodynamics due to neurovascular coupling (fNIRS, fMRI, PET), electromagnetic fields due to neural currents (MEG, EEG), eye movements (saccades) together with pupil size or blink rate (eye-tracking).
For example, integrating fNIRS and EEG provides monitoring of different events related to the same neural activity to examine cortical activity more comprehensively: EEG has a high temporal resolution, fNIRS measures changes in local cerebral blood flow that follow neural activation with a relatively high spatial resolution. Therefore, concurrent fNIRS and EEG measurements supply the research with a beneficial answer from the information content viewpoint about a cortical response to a given stimulus. Moreover, EEG and fNIRS are technologically easily attachable with one another to organize a combined measurement. The same is with combining fNIRS and eye-tracking modalities, they are not interfering with one another. Eye-tracking doesn’t provide any information about neural activation, but it’s closely connected with what is happening in human brain: intentions, expectations, beliefs, motives, and needs are connected with human brain functioning and can be detected by processing resources on what people are looking at.
To illustrate the feasibility of multimodal measurements, we provide the results from recent cognitive and development neuroscience studies. Children of the 5th grade underwent one-digit and two-digit multiplication tasks with simultaneous recordings of their fNIRS and EEG data (Soltanlou et al., 2018a). An increase in mathematics complexity while doing multiplication on the neural level is specified by activation in frontal areas (middle frontal gyrus) and theta increase together with alpha decrease, which is associated with additional demands in cognitive control, sustained attention and planning. Urakawa and colleagues (Urakawa et al., 2015) applied concurrent fNIRS and eye-tracking to investigate the infants’ neural activation together with their gaze direction during the social interactive “peek-a-boo” play with a young partner that closely resembled real-life settings. The medial prefrontal cortex (mPFC) that may play a significant role in social cognition during early infant development the same as in adults, was studied in the experiment. The results showed that hemodynamic responses significantly increased in mPFC in response to social play with a partner’s direct gaze compared to an averted gaze (Urakawa et al., 2015). The eye-tracking data revealed that the infants fixated on the partner’s eye region for a longer duration when a partner presented a direct gaze, rather than an averted gaze was, proving that looking into the eyes is a key component in social communication that forms on an early stage of human development. In general, fNIRS is stated to be well compatible with EEG, fMRI, eye-tracking, TMS, tDCS, EMG, pulse oximetry, and other modalities for concurrent measurements.
NEUROFEEDBACK TRAINING WITH FNIRS
fNIRS is considered to be a new and promising tool in neurofeedback (NF) (for a comprehensive review see Kohl et al., 2019) research. Neurofeedback is a specific type of biofeedback that provides information about certain aspects of the subjects' brain activity (Paret et al., 2019). In fNIRS neurofeedback changes in oxy-, deoxy-, and total hemoglobin are assessed in a real-time mode and participants are given feedback in the form of visual representations (thermometer, moving scale, sound/music, jingle, moving objects, virtual fire (Kohl et al., 2019). Through multiple trainings, participants learn to regulate and enhance their behavior and cognitive functions or normalize emotional states by changing the pathological brain activation patterns. fNIRS neurofeedback has been successfully applied to improve inhibitory control and attention, and decrease ADHD symptoms through training to control prefrontal brain functions in children (Marx et al., 2015) and adults (Hudak et al., 2017). Another potential application of fNIRS neurofeedback is the treatment of social anxiety (Kimmig et al., 2019), and cognitive flexibility (Li et al., 2019), motor rehabilitation (Kober et al., 2014; Fujimoto et al., 2017) in healthy populations and patients.
Various approaches are applied nowadays to facilitate significant results in the NF domain. One is an application of a special software Turbo-satori, developed by Brain Innovation, exclusively for NIRx fNIRS instruments, that provides real-time assessment of brain function in the human cerebral cortex applicable both in a lab-based as well as in open environments. Another is combining fNIRS NF training with virtual reality paradigms that allows creating a more realistic environment to provide potentially more efficient treatments (Ehlis et al., 2018). In most neuroscience experiments participants are fully aware they are watching/listening to an artificially created and prerecorded stimuli with no possibility of real interaction. In studies, that implemented immersive VR, they can interact in a created reality that much resembles natural social settings where they can influence events. Animmersive virtual reality classroom paradigm with animated students and a teacher embedded in fNIRS NF was used in recent studies (Blume et al., 2017; Hudak et al., 2017). Participants aimed to learn to control lighting in the classroom employing their dorsolateral prefrontal brain activation. This paradigm can be used for intervention studies in schoolchildren with attention-deficit/hyperactivity disorder (ADHD) (Blume et al., 2017), or healthy children displaying traits of motor hyperactivity and impulsive behavior.
Thus, virtual reality interfaces were proved to increase training efficiency by keeping subjects motivated. Virtual reality NF can become a useful tool for treating subjects with social phobias (performance situations: giving a speech or oral presentation, and/or situations involving social interaction both in the lead-up and during the event) when virtual reality can be used to create a realistic and potentially stressful social situation that can be easily controlled by an experimenter. There are studies implemented immersive VR in desktop settings to study neural correlates of flight- or drive-simulators (Takeuchi, 2000; Li et al., 2009), or game-like simulations (Izzetoglu et al., 2003). In general, NF technique with virtual reality is stated to be well accepted in both children and adult samples (Ehlis et al., 2018).
Taking into consideration fNIRS sustainability to muscle movements, combined with its portability, and ease of application, it has been proved to be a reliable neuroimaging modality for NF.
CONCLUSION
The introduction of fNIRS technique in neuroscience has considerably expanded our understanding of the neural basis of cognitive processes contributing to developmental changes, educational practice, and social cognition and interaction. A growing number of studies with the live social interaction of multiple subjects unveil brain coupling mechanisms and provide confirming evidence to neural correlates of cognition, emotional communication, perception and motor development interrelated as a complementation to well-established behavioral research. Cognitive developmental neuroscience by applying an fNIRS as a perspective tool has an opportunity now to answer important developmental questions and shed light on the neural basis of cognitive and social processes in newborns, infants and toddlers, both in a lab-based and naturalistic settings, when some other neuroimaging techniques fail to investigate them because of their limitations. Moreover, fNIRS technique continues to evolve fast and supports an evidence-based developmental and educational sciences to provide data for best practices in learning and communicating, best teaching strategies and remediation programs for mastering and improving reading, language, mathematics competences, and other cognitive skills.
Despite the current restrictions of fNIRS, such as limited penetration depth, a temporal delay in response, and strong extra-cerebral interference, the feasibility of applying fNIRS in freely moving people within relatively unrestricted and natural environments, in neurofeedback trainings for enhancing cognitive functions in a healthy population of different age groups and for rehabilitation in patients has been proved. Nowadays fNIRS measurements can be organized both with prerecorded non-live stimuli in lab settings, as well as live stimuli and outside the laboratories due to the rapid advancement of hardware and successful developments in signal quality. The availability of fNIRS portable and wearable devices that are resistant to external light and anybody movements (head and hand movements, posture changing, walking, running) may lead to exponential growth in neuroimaging domain over the next years, particularly in the domains of real-world cognition, social interaction, and educational and developmental neuroscience (Pinti et al., 2020). To sum everything up fNIRS can be regarded as a versatile and promising instrument to investigate the neural correlates of cognitive and social development from birth and over the life span within developmental cognitive neuroscience.
Список литературы
Almajidy R.K., Mankodiya K., Abtahi M., Hofmann U.G. A Newcomer’s Guide to Functional Near-Infrared Spectroscopy Experiments. IEEE Reviews in Biomedical Engineering. 2020. 13: 292–308.
Ansari D., De Smedt B., Grabner R.H. Neuroeducation. A critical overview of an emerging field. Neuroethics. 2012. 5: 105–117.
Arimitsu T., Uchida-Ota M., Yagihashi T., Kojima S., Watanabe S., Hokuto I., Ikeda K., Takahashi T., Minagawa-Kawai Y. Functional hemispheric specialization in processing phonemic and prosodic auditory changes in neonates. Front. Psychol. 2011. 2: 202.
Aslin R.N., Shukla M., Emberson L.L. Hemodynamic correlates of cognition in human infants. Annu Rev Psychol. 2015. 66: 349–379.
Azhari A., Truzzi A., Neoh M.J-Y., Balagtas J.P.M., Tan H.H., Goh P.P., Ang X.A., Setoh P., Rigo P., Bornstein M.H., Esposito G. A decade of infant neuroimaging research: What have we learned and where are we going? Infant Behav Dev. 2020. 58: 101389.
Babiloni F., Astolfi L. Social neuroscience and hyperscanning techniques: Past, present and future. NeurosciBiobehav Rev. 2014, 44: 76–93.
Bahnmueller J., Dresler T., Ehlis A.-C., Cress U., Nuerk H.-C. NIRS in motion—unraveling the neurocognitive underpinnings of embodied numerical cognition. Front. Psychol. 2014. 5: 743.
Balardin J.B., Zimeo Morais G.A., Furucho R.A., Trambaiolli L.R., Sato J.R. Impact of communicative head movements on the quality of functional near-infrared spectroscopy signals: negligible effects for affirmative and negative gestures and consistent artifacts related to raising eyebrows. J. Biomed. Opt. 2017. 22: 046010.
Blasi A., Lloyd-Fox S., Katus L., Elwell C.E. fNIRS for tracking brain development in the context of global health projects. Photonics. 2019. 6 (3): 89.
Bleichner M.G., Lundbeck M., Selisky M., Minow F., Jager M., Emkes R., Debener S., De Vos M. Exploring miniaturized EEG electrodes for brain-computer interfaces. An EEG you do not see? Physiological Reports. 2015. 3 (4): e12362.
Blume F., Hudak J., Dresler T., Ehlis A.-C., Kühnhausen J., Renner T. J., Gawrilow C. NIRS-based neurofeedback training in a virtual reality classroom for children with attention-deficit/hyperactivity disorder: study protocol for a randomized controlled trial. Trials. 2017. 18 (1): 41.
Boas D.A., Elwell C.E., Ferrari M., Taga G. Twenty years of functional near-infrared spectroscopy: Introduction for the special issue. Neuroimage. 2014. 85: 1–5.
Brigadoi S., Coope, R.J. How short is short? Optimum source – detector distance for short-separation channels in functional near-infrared spectroscopy. Neurophotonics. 2015. 2: 25005.
Brockington G., Balardin J.B., Zimeo Morais G.A., Malheiros A., Lent R., Moura L.M., Sato J.R. From the Laboratory to the Classroom: The Potential of Functional Near-Infrared Spectroscopy in Educational Neuroscience. Front. Psychol. 2018. 9: 1840.
Chance B., Zhuang Z., Un Ah C., Alter C., Lipton L. Cognition-activated low-frequency modulation of light absorption in human brain. Proc. Natl. Acad. Sci. USA. 1993. 90 (8): 3770–3774.
Chitnis D., Cooper R.J., Dempsey, L., Powell S., Quaggia S., Highton D., Elwell C., Hebden J.C., Everdell N.L. Functional imaging of the human brain using a modular, fibreless, high-density diffuse optical tomography system. Biomed Opt Express. 2016. 7: 4275.
Cui X., Bryant D.M., Reiss A.L. NIRS-based hyperscanning reveals increased interpersonal coherence in superior frontal cortex during cooperation. Neuroimage. 2012. 9 (3): 2430–2437.
Curtin A., Ayaz H. The age of neuroergonomics: Towards ubiquitous and continuous measurement of brain function with fNIRS. Japanese Psychological Research. 2018. 60: 374–386.
Curtin A., Tong S., Sun J., Wang J., Onaral B., Ayaz H. A Systematic Review of Integrated Functional Near-Infrared Spectroscopy (fNIRS) and Transcranial Magnetic Stimulation (TMS) Studies. Front. Neurosci. 2019. 13: 84.
Cutini S., Basso Moro S., Bisconti S. Functional near infrared optical imaging in cognitive neuroscience: an introductory review. Journal of Near Infrared Spectroscopy. 2012. 20 (1): 75–92.
Delpy D.T., Cope M., van der Zee P., Arridge S., Wray S., Wyatt J. Estimation of optical pathlength through tissue from direct time of flight measurement. Phys. Med. Biol. 1988. 33 (12): 1433–1442.
Eggebrecht A., Ferradal S., Robichaux-Viehoever A., Hassanpour M.S., Dehghani H., Snyder A.Z., Hershey T., Culver J.P. Mapping distributed brain function and networks with diffuse optical tomography. Nature Photonics. 2014. 8 (6): 448–454.
Ehlis A., Barth B., Hudak J., Storchak H., Weber L., Kimmig A.S., Kreifelts B., Dresler T., Fallgatter A.J. Near-infrared spectroscopy as a new tool for neurofeedback training: Applications in psychiatry and methodological considerations. Jpn. Psychol. Res. 2018. 60: 225–241.
Fantini S., Frederick B., Sassaroli A. Perspective: Prospects of non-invasive sensing of the human brain with diffuse optical imaging. APL Photonics. 2018. 3: 110901.
Fiske A., Holmboe K. Neural substrates of early executive function development. Developmental Review. 2019. 52: 42–62.
Fujimoto H., Mihara M., Hattori N., Hatakenaka M., Yagura H., Kawano T., Miyai I., Mochizuki H. Neurofeedback-induced facilitation of the supplementary motor area affects postural stability. Neurophotonics. 2017. 4 (4): 045003.
Funane T., Sato H., Yahata N., Takizawa R., Nishimura Y., Kinoshita A., Katura T., Atsumori H., Fukuda M., Kasai K., Koizumi H., Kiguchi M. Concurrent fNIRS-fMRI measurement to validate a method for separating deep and shallow fNIRS signals by using multidistanceoptodes. Neurophotonics. 2015. 2 (1): 015003.
Galderisi A., Brigadoi S., Cutini S., Moro S.B., Lolli E., Meconi F., Benavides-Varela S., Baraldi E., Amodio P., Cobelli C., Trevisanuto D., Dell’Acqua R. Long-term continuous monitoring of the preterm brain with diffuse optical tomography and electroencephalography: a technical note on cap manufacturing. Neurophotonics. 2016. 3: 045009.
Jöbsis F.F. Noninvasive, infrared monitoring of cerebral and myocardial oxygen sufficiency and circulatory parameters. Science. 1977. 198: 1264–1267.
Herold F., Wiegel P., Scholkmann F., Thiers A., Hamacher D., Schega L. Functional near-infrared spectroscopy in movement science: A systematic review on cortical activity in postural and walking tasks. Neurophoton. 2017. 4: 41403.
Hoshi Y., Tamura M. Detection of dynamic changes in cerebral oxygenation coupled to neuronal function during mental work in man. Neurosci. Lett. 1993. 150 (1): 5–8.
Hudak J., Blume F., Dresler T., Haeussinger F.B., Renner T.J., Fallgatter A.J., Gawrilow C., Ehlis A.C. Near-infrared spectroscopy-based frontal lobe neurofeedback integrated in virtual reality modulates brain and behavior in highly impulsive adults. Frontiers in Human Neuroscience. 2017. 11: 425.
Hyde D.C., Simon C.E., Ting F., Nikolaeva J.I. Functional organization of the temporal–parietal junction for theory of mind in preverbal infants: A near-infrared spectroscopy study. Journal of Neuroscience. 2018. 38: 4264–4274.
Kimmig A.-C.S., Dresler T., Hudak J., Haeussinger F.B., Wildgruber D., Fallgatter A.J., Ehlis A.C., Kreifelts B. Feasibility of NIRS-based neurofeedback training in social anxiety disorder: behavioral and neural correlates. Journal of Neural Transmission. 2019. 126 (9): 1175–1185.
Kober S.E., Wood G., Kurzmann J., Friedrich E.V.C., Stangl M., Wippel T., Väljamäe A., Neuper C. Near-infrared spectroscopy based neurofeedback training increases specific motor imagery related cortical activation compared to sham feedback. Biol. Psychol. 2014. 95: 21–30.
Kohl S.H., Mehler D.M.A., Lührs M., Thibault R.T., Konrad K., Sorger B. The potential of functional near-infrared spectroscopy-based neurofeedback – a systematic review and recommendations for best practice. Front Neurosci. 2020. 14: 594.
Koike T., Tanabe H.C., Sadato N. Hyperscanning neuroimaging technique to reveal the two-in-one system in social interactions. Neuroscience Research. 2015. 90: 25–32.
Lauritzen M., Gold L. Brain function and neurophysiological correlates of signals used in functional neuroimaging. J Neurosci. 2003. 23.
Leff D.R., Orihuela-Espina F., Elwell C.E., Athanasiou T., Delpy D.T., Darzi A.W., Yang G.Z. Assessment of the cerebral cortex during motor task behaviors in adults: A systematic review of functional near-infrared spectroscopy (fNIRS) studies. NeuroImage. 2011. 54 (4): 2922–2936.
León-Carrión J., León-Domínguez U. Functional near-infrared spectroscopy (fNIRS): Principles and neuroscientific applications. Neuroimaging Methods. Prof. Peter Bright (Ed.), 2012. ISBN: 978-953-51-0097-3, InTech, Available from: http://www.intechopen.com/books/neuroimaging-methods/functional-nearinfrared-spectroscopy-fnirs-brain-studies-and-others-clinical-uses
Liao S.M., Culver J.P. Near-infrared optical technologies to illuminate the status of the neonatal brain. Current Pediatric Reviews. 2014. 10 (1): 73–86.
Liu Y., Piazza E.A., Simony E., Shewokis P.A., Onaral B., Hasson U., Ayaz H. Measuring speaker – listener neural coupling with functional near-infrared spectroscopy. Sci. Rep. 2017. 7:43293.
Lloyd-Fox S., Begus K., Halliday D., Pirazzoli L., Blasi A., Papademetriou M., Darboe M.K., Prentice A.M., Johnson M.H., Moore S.E., Elwell C.E. Cortical specialization to social stimuli from the first days to the second year of life: A rural Gambian cohort. Dev Cogn Neurosci. 2016. 25: 92–104.
Lloyd-Fox S., Blasi A., Elwell C.E. Illuminating the developing brain: the past, present and future of functional near infrared spectroscopy. Neurosci Biobehav Rev. 2010. 34: 269–84.
Lloyd-Fox S., Blasi A., Elwell C.E., Charman T., Murphy D., Johnson M.H. Reduced neural sensitivity to social stimuli in infants at risk for autism. Proc. R. Soc. B Biol. Sci. 2013. 280.
Lloyd-Fox S., Papademetriou M., Darboe M.K., Everdell N.L., Wegmuller R., Prentice A.M., Moore S.E., Elwell C.E. Functional near-infrared spectroscopy (fNIRS) to assess cognitive function in infants in rural Africa. Sci. Rep. 2014. 4: 4740.
McDonald N.M., Perdue K.L. The infant brain in the social world: Moving toward interactive social neuroscience with functional near-infrared spectroscopy. Neuroscience and Biobehavioral Reviews. 2018. 87: 38–49.
Marx A.M., Ehlis A.C., Furdea A., Holtmann M., Banaschewski T., Brandeis D., Rothenberger A., Gevensleben H., Freitag C.M., Fuchsenberger Y., Fallgatter A.J., Strehl U. Near-infrared spectroscopy (NIRS) neurofeedback as a treatment for children with attention deficit hyperactivity disorder (ADHD): A pilot study. Frontiers in Human Neuroscience. 2015. 8 (1038).
Matusz P.J., Dikker S., Huth A.G., Perrodin C. Are We Ready for Real-world Neuroscience? Journal of Cognitive Neuroscience. 2019. (3): 327–338.
Mehnert J., Akhrif A., Telkemeyer S., Rossi S., Schmitz C.H., Steinbrink J., Wartenburger I., Obrig H., Neufang S. Developmental changes in brain activation and functional connectivity during response inhibition in the early childhood brain. Brain Dev. 2012. 35 (10): 894–904.
Minagawa Y., Xu M., Morimoto S. Toward interactive social neuroscience: Neuroimaging real-world interactions in various populations. The Japanese Psychological Research. 2018. 60 (4): 196–224.
Moriguchi Y., Sakata C. Development of Cognitive Shifting from Others’ Behavior in Young Children: A Near-infrared Spectroscopy Study. Developmental Neuropsychology. 2019. 1–9.
Morita T., Asada M., Naito E. Contribution of Neuroimaging Studies to Understanding Development of Human Cognitive Brain Functions. Front. Hum. Neurosci. 2016. 10: 464.
Munakata Y., Casey B. J., Diamond A. Developmental cognitive neuroscience: progress and potential. Trends Cogn. Sci. 2004. 8; 122–128.
Nagamitsu S., Yamashita Y., Tanaka H., Matsuishi T. Functional near-infrared spectroscopy studies in children. Biopsychosocial Medicine 2012. 6(1): 7.
Orihuela-Espina F., Leff D.R., James D.R.C., Darzi A.W., Yang G.Z. Quality control and assurance in functional near-infrared spectroscopy (fNIRS) experimentation Phys. Med. Biol. 2010. 55 (13): 3701–3724.
Paret C., Goldway N., Zich C., Keynan J.N., Hendler T., Linden D., Kadosh K.C. Current progress in real-time functional magnetic resonance-based neurofeedback: Methodological challenges and achievements. Neuroimage. 2019. 116107.
Patil A.V., Safaie J., Moghaddam H.A., Wallois F., Grebel R. Experimental investigation of NIRS spatial sensitivity. Biomed. Opt. Express. 2011. 2: 1478–1493.
Pfeifer M.D., Scholkmann F., Labruyère R. Signal processing in functional near-infrared spectroscopy (fNIRS): Methodological differences lead to different statistical results. Front. Hum. Neurosci. 2018. 11: 20801.
Pinti P., Aichelburg C., Gilbert S., Hamilton A., Hirsch J., Burgess P., Tachtsidis I. A review on the use of wearable functional near-infrared spectroscopy in naturalistic environments. Japanese Psychological Research. 2018a. 60: 347–373.
Pinti P., Aichelburg C., Gilbert S., Hamilton A., Hirsch J., Burgess P., Tachtsidis I. A review on the use of wearable functional near-infrared spectroscopy in naturalistic environments. Jpn. Psychol. Res. 2018, 2, 20801.
Pinti P., Aichelburg C., Lind F., Power S., Swingler E., Merla A., Hamilton A., Gilbert S., Burgess P., Tachtsidis I. Using fiberless, wearable fNIRS to monitor brain activity in real-world cognitive tasks. Journal of Visualized Experiments. 2015. 106: 53336.
Pinti P., Tachtsidis I., Hamilton A., Hirsch J., Aichelburg C., Gilbert S., Burgess P.W. The present and future use of functional near-infrared spectroscopy (fNIRS) for cognitive neuroscience. Ann NY Acad Sci. 2020 Mar; 1464 (1): 5–29. Epub 2018 Aug 7.https://doi.org/10.1111/nyas.13948
Quaresima V., Ferrari M. Functional near-infrared spectroscopy (fNIRS) for assessing cerebral cortex function during human behavior in natural/social situations: A concise review. Organ. Res. Methods 2019a. 22: 46–68.
Quaresima V., Ferrari M. A Mini-Review on Functional Near-Infrared Spectroscopy (fNIRS): Where Do We Stand, and Where Should We Go? Photonics. 2019b. 6: 87.
Reindl V., Gerloff C., Scharke W., Konrad K. Brain-to-brain synchrony in parent-child dyads and the relationship with emotion regulation revealed by fNIRS-based hyperscanning. Neuroimage. 2018. 178: 493–502.
Rupawala M., Dehghani H., Lucas S.J.E., Tino P., Cruse D. Shining a light on awareness: a review of functional near-infrared spectroscopy for prolonged disorders of consciousness. Front. Neurol. 2018 9: 350.
Scarapicchia V., Brown C., Mayo C., Gawryluk J.R. Functional magnetic resonance imaging and functional near-infrared spectroscopy: insights from combined recording studies. Front. Hum. Neurosci. 2017. 11: 419.
Schneider S., Christensen A., Haußinger F.B., Fallgatter A.J., Giese M. A., Ehlis A.C. Show me how you walk and I tell you how you feel – A functional near-infrared spectroscopy study on emotion perception based on human gait. Neuroimage. 2014. 85 (1): 380–390.
Scholkmann F., Holper L., Wolf U., Wolf M. A new methodical approach in neuroscience: Assessing inter-personal brain coupling using functional near-infrared imaging (fNIRI) hyperscanning. Frontiers in Human Neuroscience. 2013. 7: 813.
Scholkmann F., Kleiser S., Metz A.J., Zimmermann R., Pavia J.M., Wolf U., Wolf M. A review on continuous wave functional near-infrared spectroscopy and imaging instrumentation and methodology. Neuroimage 2014. 85: 6–27.
Shamay-Tsoory S.G., Mendelsohn A. Real-Life Neuroscience: An Ecological Approach to Brain and Behavior Research. Perspectives on Psychological Science. 2019. 174569161985635.
Soltanlou M., Artemenko C., Ehlis A.-C., Huber S., Fallgatter A.J., Dresler T., Nuerk H-C. Reduction but no shift in brain activation after arithmetic learning in children: a simultaneous fNIRS-EEG study. Sci. Rep. 2018a. 8: 1707.
Soltanlou M., Sitnikova M.A., Nuerk H-C., Dresler T. Applications of Functional Near-Infrared Spectroscopy (fNIRS) in Studying Cognitive Development: The Case of Mathematics and Language. Front. Psychol. 2018b. 9: 277.
Steinmayr R., Ziegler M., Trauble B. Do intelligence and sustained attention interact in predicting academic achievement? Learn. Indiv. Differ. 2010. 20, 14–18.
Strangman G.E., Li Z., Zhang Q. Depth sensitivity and source-detector separations for near-infrared spectroscopy based on the Colin27 brain template. PLoS ONE 2013. 8: e 66319.
Suda M., Takei Y., Aoyama Y., Narita K., Sato T., Fukuda M., Mikuni M. Frontopolar activation during face-to-face conversation: An in situ study using near-infrared spectroscopy. Neuropsychologia. 2010. 48 (2): 441–447.
Tellis G., Tellis C. Using functional near-infrared spectroscopy with fluent speakers to determine hemoglobin changes in the brain during speech and non-speech tasks. NIR News. 2016. 27: 4–7.
Urakawa S., Takamoto K., Ishikawa A., Ono T., Nishijo H. Selective medial prefrontal cortex responses during live mutual gaze interactions in human infants: an fNIRS study. Brain Topogr. 2015. 28: 691–701.
Vannasing P., Florea O., González-Frankenberger B., Tremblay J., Paquette N., Safi D., Wallois F., Lepore F., Béland R., Lassonde M., Gallagher A. Distinct hemispheric specializations for native and non-native languages in one-day-old newborns identified by fNIRS. Neuropsychologia. 2016. 84: 63–69.
Vanderwert R.E., Nelson C.A. The use of near-infrared spectroscopy in the study of typical and atypical development. NeuroImage. 2014. 85(1): 264–271.
Villringer A., Planck J., Hock C., Schleinkofer L., Dirnagl U. Near-infrared spectroscopy (NIRS): a new tool to study hemodynamic changes during activation of brain function in human adults. Neurosci. Lett. 1993. 154 (1–2): 101–104.
Wilcox T., Biondi M. fNIRS in the developmental sciences. Wiley Interdisciplinary Review, Cognitive Science. 2015. 6 (3): 263–283.
Yücel M.A., Selb J.J., Huppert T.J., Franceschini M.A., Boas D.A. Functional near-infrared spectroscopy: Enabling routine functional brain imaging. Curr. Opin. Biomed. Eng. 2017. 4: 78–86.
Дополнительные материалы отсутствуют.
Инструменты
Журнал высшей нервной деятельности им. И.П. Павлова